INTRODUCTION
In the field of medicine, the use of animal models is essential for preclinical evaluation of drugs and therapies. Each of the various animal models offers its own advantages and disadvantages, and use of rodent models, such as mice, which are easy to breed and maintain, has been the preference. Increasing use of pigs, known for having anatomical, physiological, and biochemical characteristics most similar to humans, as animal models has been reported. In particular, mini-pigs have emerged as an important animal model for assessing toxicity and in conduct of pharmaceutical research.
Although drugs can be administered through various routes, interest in intradermal administration as an alternative to conventional subcutaneous, intramuscular, or intravenous methods is on the rise, owing to its minimal invasiveness, reduced pain, rapid pharmacokinetics, and the presence of a high density of antigen-presenting cells and blood vessels. Successful intradermal administration is based on the presence of a small visible wheal on the surface of skin. Although commonly used for administration of a tuberculosis vaccination, intradermal administration, which can be technically challenging, is significantly influenced by the practitioner's experience and the biomechanical properties of the skin [1]. Devices that included pen injectors, microinjection equipment, and needle-free injection systems have been developed in recent years to enhance the accuracy and ease of intradermal administration [2]. Some have been commercialized, while others are still under development. Effective delivery of vaccines and drugs through intradermal administration requires optimization of the drug composition along with administration-related equipment. Ideally, this process of optimization should occur in animal models or in vitro skin models during the early stages of drug or device development.
Utilization of large animals is often required when performing these types of procedures. Culturing conditions that preserve protein expression in samples even after euthanasia were identified. Images of the sample plates were captured using small animal imaging machines. This research represents a significant contribution to the advancement of methods for bioluminescence imaging (BLI) in large animal models, which haven gained importance in the fields of drug development and biomedical research.
MATERIALS AND METHODS
QuantiLum® Recombinant Luciferase (Catalog #E1701, Promega, Madison, WI, USA), a custom-prepared imaging buffer (IB), and luciferase for detection of Fluc protein luminescence (Catalog #P1043, Promega) were mixed and added to 96-well plates, to a volume of 100 µL per well. The IB was composed of 30 mM HEPES pH 7.4, 1 mM β-mercaptoethanol, 1 mM adenosine triphosphate (ATP) pH 7.4, 1 mM potassium luciferin, 10 mM MgSO4, and 1 mg/mL bovine serum albumin; the concentration of luciferase was set at 21 µL/mL. After dispensing 100 µL of the mixture into each well, imaging was performed using imaging equipment acquired from DaVinci (Seoul, Korea) in ultra mode at 1-second intervals for 10 seconds. Analysis of the imaging was performed using custom software within the imaging equipment.
Female SD rats aged six weeks (RaonBio, Yongin, Korea) were used; anesthesia was administered via inhalation using Isoflurane (DaonPharm, Seongnam, Korea) during administration and imaging. QuantiLum® Recombinant Luciferase (E1701, Promega) was prepared at concentrations of 0, 12.5, 25, 50, 100, 200, 400, 800, 1,600, and 3,200 ng with addition of 0.125 mg/mL Food green 3 (FCF) dye (F7252, Sigma-Aldrich, St. Louis, MO, USA) in phosphate-buffered saline (PBS) and mixed with a custom-made IB to a total volume of 50 µL, and the mixture was incubated at 37℃ for 30 minutes before intradermal administration. At 20 minutes post-administration, an intraperitoneal injection of luciferin (P1043, Promega) was administered at a concentration of 150 mg/kg, followed by performance of imaging 10 minutes later. Imaging was performed in fast mode at 30-second intervals for 2 minutes. Analysis of imaging was performed using custom software within the imaging equipment.
Seven-month-old, 15 kg male mini-pigs (mini-pig, Endic, Gwangju, Korea; Aphrodite's micropig, Pyeongtaek, Korea) were used in the experiment. Fluc protein was administered at varying concentrations 0, 400, 1,600, and 6,400 with addition of 0.125 mg/mL FCF dye in PBS at a volume of 50 µL on the back region. For 1-site injections, the entire 50 µL was administered at one location, while for 4-site injections, the 50 µL volume was divided and administered across four locations. Immediately following administration, euthanasia was performed, and an 8 mm biopsy punch (Kai Medical, Seki, Japan) was performed; 1 mL of DP BS was added to an E-tube containing the sample, which was then transported to Hulux, while a temperature of 4℃ was maintained, which took approximately 2 hours. A 12-well plate with a transparent membrane (pore diameter: 1 µm, Greiner Bio-One, Kremsmünster, Austria) was prepared, followed by addition of 1 mL of media and 0.1 mL of luciferin (3 mg/mL) to each well in anticipation of the samples. The 8 mm punch samples were placed in each well. Imaging was performed approximately 3 hours after administration of luciferase to allow for sufficient absorption. Imaging was performed in ultra mode; images were taken at 10-second intervals over a period of 2 minutes. Analysis of the imaging was performed using proprietary software within the imaging equipment.
RESULTS
The following conditions were used to evaluate ex vivo expression of rFluc: #1 = 0, #2-1, #2-2 = 0.61 µL/mL, #3 = 1.22 µL/mL, #4 = 2.44 µL/mL. Added to each well of a 96-well plate, experiments were performed according to the following specifications: concentrations of #1 = 0, #2-1, #2-2 = 0.61 µL/mL, #3 = 1.22 µL/mL, and #4 = 2.44 µL/mL. Following addition of 100 µL of PBS to each well of a 96-well plate, the specified concentrations of rFluc were added, followed by administration of luciferin at a concentration of 21 µL/mL imaging performed at 1-second intervals for 10 seconds showed that the highest concentration, #4, began to emit light after 5 seconds (Fig. 1A and B). We examined the question of whether the luminescence of rFluc could be enhanced by addition of a custom-made IB. When IB was included under the same conditions as outlined in Fig. 1A, luminescence was observable at all concentrations starting from the first second and was sustained for up to 10 seconds (Fig. 1C and D). Based on these experimental results, the establishment of IB buffer conditions not only facilitates performance of ex vivo luminescence experiments but also offers the potential for application of these conditions in animal studies.
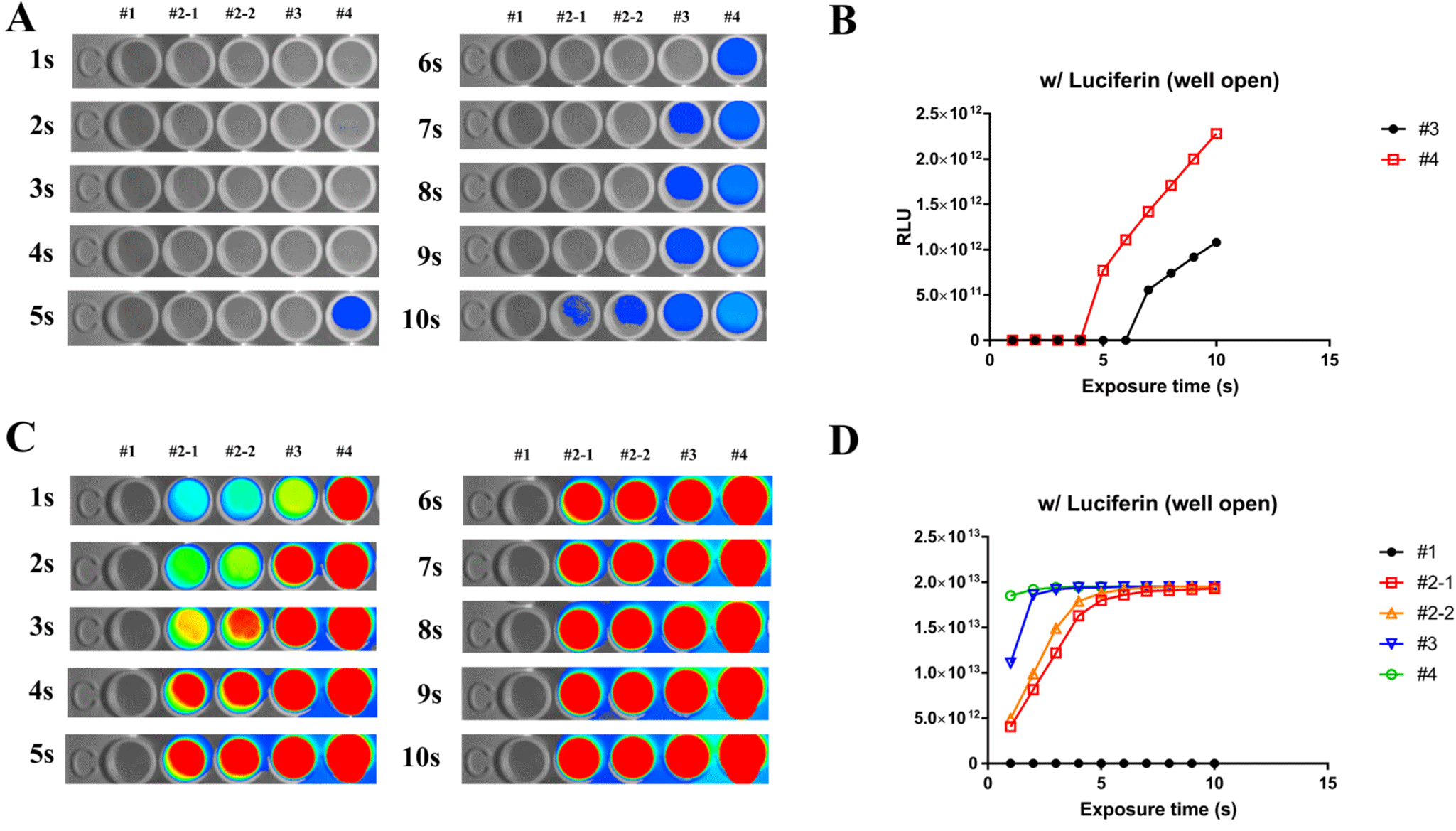
To confirm the luminescence of rFluc in live rats, a reaction time is required prior to administration of luciferin to ensure expression within the body. Before entry of protein into the cell, minimal necessary components including ATP must be supplied to initiate activity within a short time outside the cells [3-6]. Using the established IB shown in Fig. 1, luminescence was observable within 1 hour after administration (Fig. 2A). In 6-week-old female SD rats, varying concentrations of rFluc (0, 12.5, 25, 50, 100, 200, 400, 800, 1,600, and 3,200 ng with the addition of 0.125 mg/mL FCF dye) were mixed with the custom-made IB to a total volume of 50 µL, followed by incubation at 37 degrees Celsius for 30 minutes and then intradermal administration into the peritoneal cavity. Imaging performed in fast mode with a 5-minute exposure time showed that the luminescence of rFluc increased in a concentration-dependent manner (Fig. 2A and B).
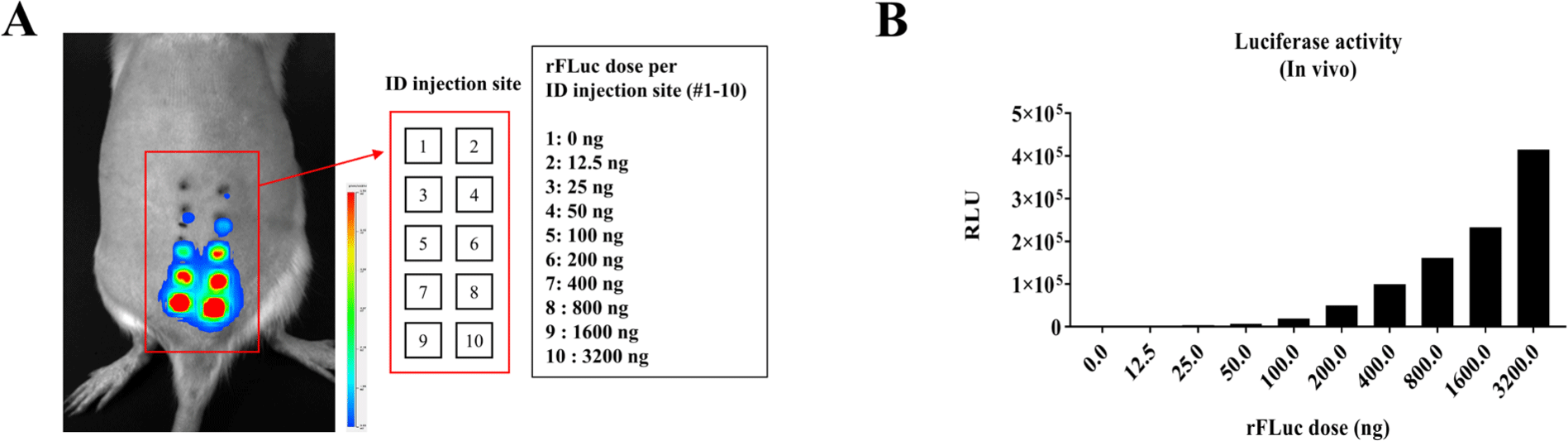
Luminescence of proteins can be rapidly confirmed ex vivo and in small animals in vivo, however, verifying luminescence using imaging equipment is challenging due the larger size of large animals, as opposed to small animals. In addition, after the death of an organism, the absence of essential components such as ATP, even after administering luciferin and waiting for the reaction time, can complicate the confirmation of rFluc expression and luminescence. Therefore, by integrating the findings shown in Figs. 1 and 2, conditions that enable measurement of rFluc luminescence ex vivo in large animals were identified. Using mini-pig skin, preparations were made in a 12-well plate under the conditions shown in Fig. 3A, and doses of 800, 1200, 1,600, and 2,000 ng of rFluc were mixed with the IB to a total volume of 50 µL prior to administration (Fig. 3B). Imaging was performed 3 hours after administration of luciferin, following the reaction time. ex vivo administration of the protein into skin tissue confirmed luminescence at varying concentrations (Fig. 3C and D). Based on these experimental outcomes, the conditions for rFluc luminescence using large animal skin tissue were established.
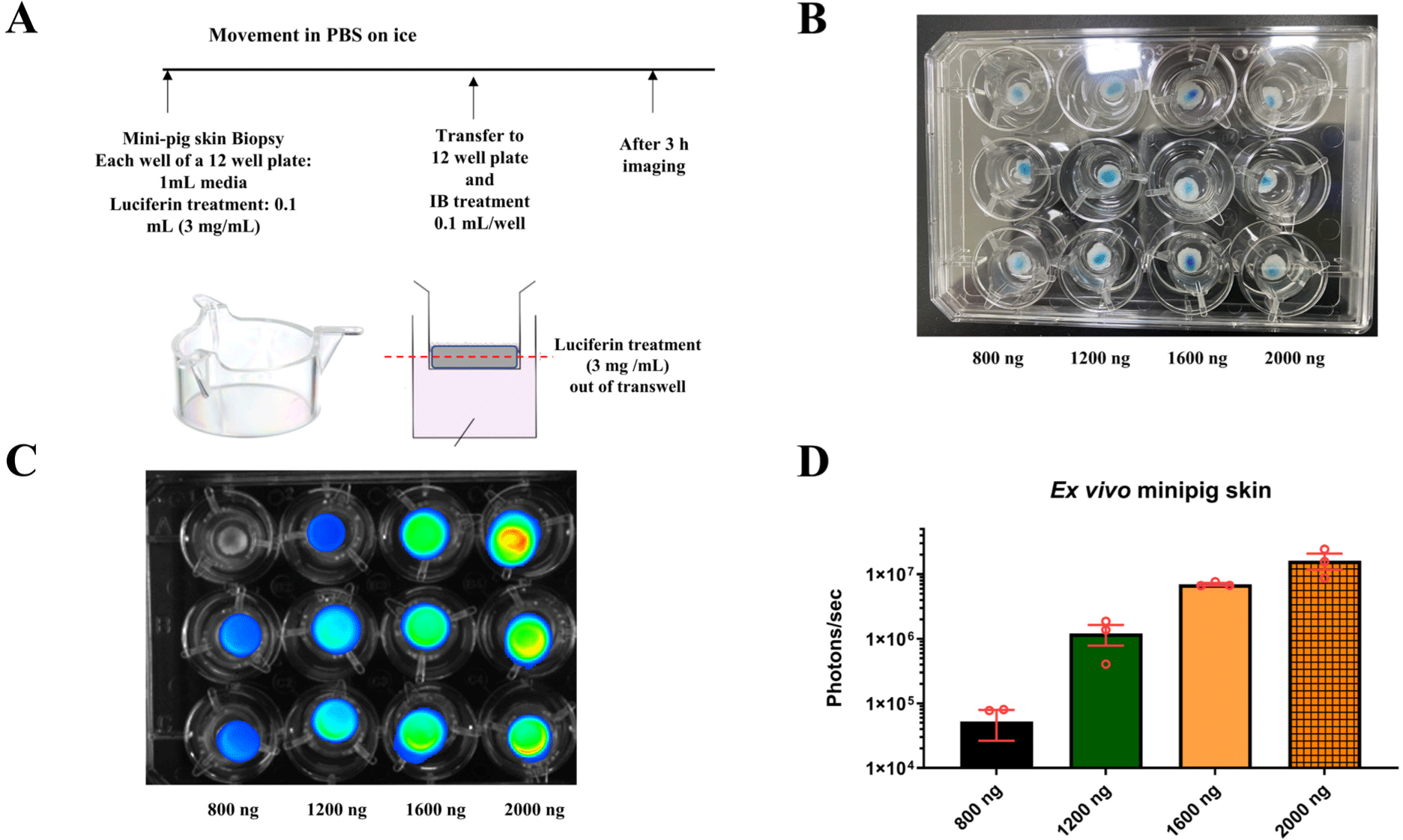
The established conditions for rFluc luminescence were further validated ex vivo in large animal models. Specifically, the rFluc protein was administered at concentrations of 0, 400, 1,600, and 6,400 ng in a 50 µL volume to the dorsal region of 7-month-old, 15 kg male mini-pigs (Fig. 4A and C). The strategy for administration was differentiated based on the use of two approaches: a single-site (1 site) treatment, where the entire dose of 50 µL was injected at one specific location (Fig. 4B), and a multiple-site (4 site) treatment, where the 50 µL dose was divided equally and administered across four distinct locations (Fig. 4D). This outcome not only corroborates the effectiveness of the custom-made IB in enhancing luminescence but also demonstrates the feasibility of applying these optimized conditions for precise and controlled evaluation of protein expression in large animal skin tissues, as previously established through conduct of ex vivo experiment using mini-pig skin (Fig. 3). Use of both experimental setups resulted in a concentration-dependent increase in rFluc luminescence. The findings from use of this integrated approach emphasize the potential for use of our methodology for broad applications in biomedical research involving large animal models.
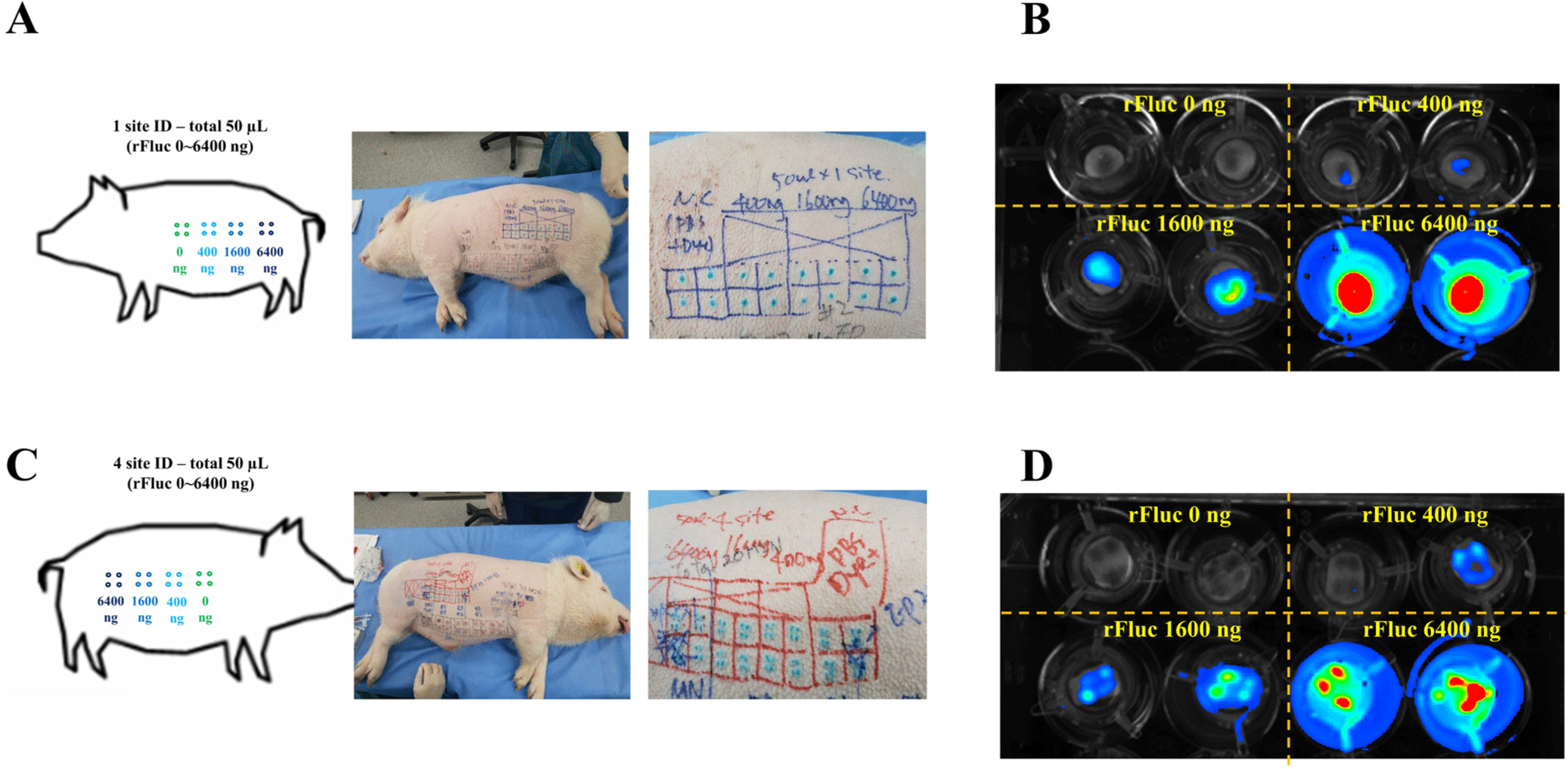
DISCUSSION
In current biological research, application of in vivo BLI as a method has shown a steady increase [7]. As a result of advancements in BLI techniques, there is significant potential for the refinement of drug development processes and evaluation of preclinical models [8]. Due to its cost-effectiveness, high throughput, and ease of operation for visualizing a broad range of in vivo cellular events, researchers from diverse disciplines are adopting the use of whole-animal BLI [9]. Implementation of BLI is easy and can facilitate continuous monitoring during the progression of the disease, enabling localization and serial quantification of biological processes without sacrificing the experimental animal [10, 11]. Introduction of a custom-made IB has resulted in enhanced luminescence of rFluc, enabling precise and rapid validation of the levels of protein expression both ex vivo and in vivo across different animal models [12].
The successful application of these techniques in small animals, particularly rats, highlights the potential for use of BLI in conduct of in-depth pharmacokinetic and pharmacodynamic studies [13]. These types of studies are critical in the effort to understand the efficacy and safety profiles of new therapeutic agents before progression to human clinical trials. In addition, in our work we have extended the use of these applications to large animals, including mini-pigs, who share closer physiological and anatomical similarities with humans [14]. Thus, they are considered invaluable for conduct of translational research, where findings from animal studies can be applied more directly to human medicine [14].
Extrapolating findings from studies using small animals to the human situation, which may not accurately reflect human physiology and/or dimensional conditions, can be challenging [15]. Conversely, the dimensions of large mammals hinder performance of in vivo BLI due to the limited penetration depth of BLI. Utilization of BLI in ex vivo organ culture systems may be considered as a potential solution in the effort to address this issue [16, 17].
The technical challenges of using BLI in large animals, primarily due to their size and the post-mortem absence of essential components such as ATP [18], were addressed by adapting the conditions confirmed in our rat model studies. The key components of BLI include light-generating luciferase enzymes, including firefly luciferase, Renilla luciferase, Gaussia luciferase, Metridia luciferase, Vargula luciferase, or bacterial luciferase [19-22]. A buffer formulation for ex vivo BLI was developed after a review of literature for experiments in large animals. This adaptation facilitated assessment of rFluc luminescence in large animal skin tissues, offering a novel approach for evaluating protein expression and drug efficacy in models that are more representative of human patients.
In conclusion, our findings contribute to the growing body of research supporting expanded use of BLI in conduct of both small and large animal studies. Establishing optimized conditions for rFluc luminescence and demonstrating the feasibility of these techniques in large animal models can enable performance of more accurate and efficient preclinical evaluations. Future research should focus on further refining these techniques, exploring their applications in other large animal models, and development of standardized protocols for their use in drug development and biomedical research.