Introduction
Aluminum (Al) is one of the most abundant metals found in the earths’ crust. Numerous industries use Al as their primary input metal. The metallic form of Al is mainly used in vehicles, packaging materials, and construction sites, whereas the non-metallic Al salts are applied in the processed food and medical sector [1]. Rapid industrialization has escalated the risk of environmental pollution, with the continuous daily input resulting in increased metal concentration in the natural environment. Improper dumping of industrial waste products directly into the aqueous and terrestrial areas is one of the main problems encountered in the modern world [2]. Apart from anthropogenic activities, the weathering of rocks and minerals increases the Al levels in the natural environment [3]. This, in turn, increases human exposure to Al, and high concentrations of Al are known to be toxic to living bodies. They especially act as a neurotoxin, inducing various neurodegenerative diseases such as Alzheimer’s disease, Parkinsonism-dementia, and amyotrophic lateral sclerosis [4–6]. Although past literature has validated that excess Al promotes adverse health effects in living bodies, more studies are required for full understanding.
To conduct toxicological studies, the quest to identify a fitting non-mammalian animal model became a necessity due to the complex ethical concerns regarding laboratory mammalians. Among certain invertebrate species, planarians have gained considerable attention over the past years as an alternative animal model. Planarians are free-living flatworms that naturally inhabit in rivers, streams, and ponds. They are simple organisms comprising three tissue layers and having bilateral symmetry of the central nervous system. Moreover, their photoreceptors as well as chemoreceptors are located in the head region [7]. Most importantly, their anatomical and physiological nature makes them most suitable for toxicity studies. Planarians have a short life cycle and both sexual and asexual reproduction forms, which are beneficial features for researchers [8]. Furthermore, the development and regeneration of the body occurs approximately within one week, thereby rendering the observation of regeneration to be conducted within a short period [9]. The same study also states that the brain of planarians has structural and molecular similarity to the mammalian brain. Apart from their beneficial physiological and biological characteristics, planarians are very easy to handle, have a low maintenance cost, and are easy to manage. Due to their comparatively high sensitivity towards environmental chemicals, planarians are a promising screening tool for toxicity studies related to mammals [10, 11]. As mentioned earlier, rapid industrialization has resulted in increased human exposure to Al, thereby making it imperative to evaluate the toxic effects. This has necessitated finding a suitable animal species, since toxicity studies with laboratory mammalians have numerous ethical barriers and are comparatively more expensive. The present study therefore aims to determine Al toxicity on planarians, while evaluating their suitability as an animal model for toxicity studies.
Materials and Methods
Planarian (Dugesia japonica) was used in this current study. They were first acclimated in freshwater at 18°C, and fed homogenized beef liver twice a week. Planarians were subsequently starved for one week prior to the experiment, to create a uniform metabolic state within them. Approximately 1.0 cm long planarians were used for the experiments.
Al sulfate octadecahydrate (Al2(SO4)3·18H2O; Al) was purchased from Sigma-Aldrich (St. Louis, MO, USA). Varying concentrations (50–1,200 mg/L Al) were prepared by dissolving Al in distilled water (DW).
The effect of Al on the motility and seizure-like behaviors were evaluated by applying the methods proposed by Raffa, with slight modifications [12, 13]. Briefly, planarians were introduced into 10 mL of respective treatment solutions and incubated for 1 hr. After the incubation period, planarians were transferred to new petri-dishes filled with fresh water (22°C), and these dishes were placed on a graph paper having gridlines at 0.5 cm spaced intervals. Measurements were taken by counting the number of grid lines crossed or re-crossed by each planarian within 5 min of introduction. Simultaneously, seizure-like behaviors were also evaluated for 5 min.
The head region of the treated planarians was harvested and observed under transmission electron microscopy. Briefly, planarians were fixed overnight at 4°C in a primary fixation buffer (2% paraformaldehyde + 2% glutaraldehyde in 0.05 M sodium cacodylate buffer [pH 7.2]). Specimens were subsequently washed 3 times for 10 min each, using 0.05 M sodium cacodylate buffer at 4°C. The washed specimens were fixed in the post-fixation buffer (1% OsO4 in 0.05 M sodium cacodylate buffer) for 90 min at 4°C, followed by 2 washes using filtered 3rd DW at room temperature. Specimens were then stained with a solution prepared by dissolving 0.5% uranyl acetate in 0.05 M sodium cacodylate buffer, overnight at 4°C, subsequently dehydrated through a graded series of ethanol concentrations from 50% to 100% at room temperature for 10 min, after which they were embedded in 100% propylene oxide for 15 min at room temperature. Thereafter the specimens were embedded in Embed 812 resin mixture and polymerized for 48 hr at 60°C. Observations were done under a Hitachi electron microscope, model H-7650 (Hitachi, Kyoto, Japan), and images were obtained at a magnification of 10,000 to 50,000 ×.
Regeneration assay was set up with a minimum number (n = 10) of planarians for each treatment group. They were starved for at least one week before initiating the experiments. The planarians were paralyzed by chilling on ice, and stretched bodies were cut using an ethanol-sterilized blade. Each amputated fragment was transferred to a 12-well plate filled with the treatment solutions, and maintained in a dark environment at 18°C–22°C. The imaging process of the regeneration fragments was conducted for 14 days, using a stereo-microscope (S8APO, Leica, Wetzlar, Germany).
Immunofluorescence staining was achieved by applying a previously described method, with slight modification [14]. Briefly, regenerating planarians were treated with 2% HCl (diluted in DW) for 5 min to remove excess mucus, and fixed in Carnoy’s solution (ethanol : chloroform : acetic acid = 6 : 3 : 1) for 3 hr at room temperature. Thereafter, samples were rinsed with 100% methanol and bleached overnight in 6% H2O2 diluted in methanol, under a light-emitting diode (LED) lamp at room temperature. Bleached samples were rehydrated through a graded methanol series (75%, 50%, and 25%) diluted in phosphate buffer saline (PBS), for 15 min each. Samples were subsequently rinsed in PBS containing 0.3% Triton X-100 (PBST; Sigma-Aldrich) and blocked for 2 hr in PBST containing 0.25% BSA (PBSTB; Gen-DEPOT), followed by overnight incubation at 4°C with the primary antibody, rabbit polyclonal planarian arrestin antibody (anti-arrestin, Cat. 016-arrestin-01, LAgen Laboratories, 1:5,000 dilution) in PBSTB. Probed samples were washed 3 times with PBST for 2 hr, after which the samples were incubated overnight in the dark at 4°C with Alex488-labeled secondary anti-rabbit-IgG (Invitrogen, Carlsbad, CA, USA) diluted 1:400 in PBSTB. Thereafter, samples were washed for several hours in PBST, followed by mounting on glass slides and observed under a fluorescence microscope (Nikon Eclipse Ci microscope; Nikon Instruments, Seoul, Korea).
Results
To evaluate the effect of Al toxicity, planarians were exposed to varying Al concentrations for 1–5 hr (Fig. 1). The solubility of Al in the solid phase is dependent on the pH [15], which is related to Al concentration and increasing toxicity [16]. Considering this, the pH of each solution was determined and correlated to the Al addition concentration. Water adjusted to pH 7.5, 5.5, and 4.5 (without Al) was used as the control groups. Motility was observed to significantly decrease in a dose-dependent manner after 1 hr incubation (p<0.01, p<0.001; Fig. 1A). After further incubation from 3–5 hr, similar decreasing motility patterns were observed (p<0.01, p<0.001; Fig. 1B, C). There was no statistical significance of motility in the planarians exposed to 50 and 100 mg/L Al for 1–5 hr of incubation, but the motility was significantly decreased in higher concentration of Al (500–1,200 mg/L). This can be predicted that the toxicity of Al to motility is immediately displayed at high concentrations rather than exposed time (Fig. 1). In the examination of seizure-like behavior, significantly higher numbers of c-like behavior were observed in planarian exposed to higher concentrations of Al (100–1,200 mg/L) during 1–5 hr incubation (p<0.05, p<0.01, p<0.001; Fig. 2A–C). Frequencies of head-bop behaviors were also increased in planarians incubated with Al for 1–5 hr incubations, as compared to controls (p<0.05 and p<0.01; Fig. 2A–C). The snake-like and screw-like behaviors were observed when planarians were incubated in the presence of Al, but did not appear to be significantly higher in numbers (Fig. 2A–C). Taken together, these results validate that planarians are sensitive to Al toxicity.
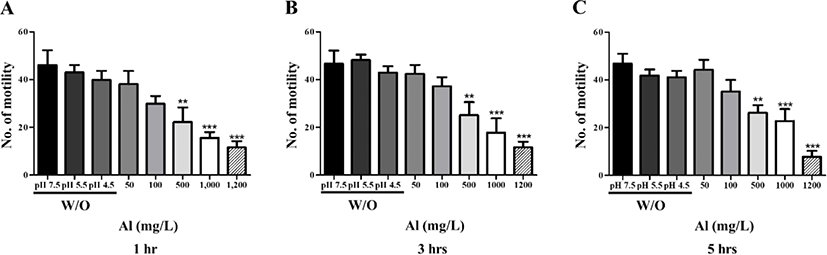
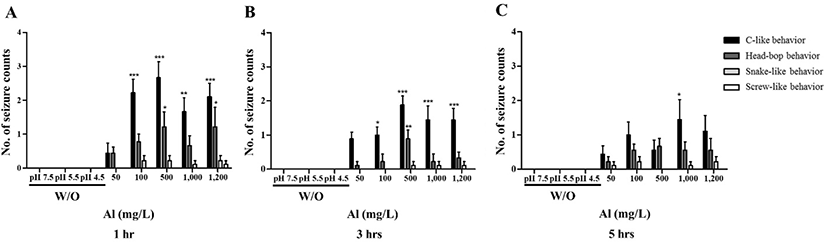
Regeneration observations were conducted by amputating the body and incubating in water at 18°C until emergence of the eye extrusion. The degree of regeneration was calculated by scoring of 0, 0.5, and 1, indicating burst, regeneration, and completion of regeneration, respectively (Fig. 3A). Compared to the treatment groups, only the control group (absence of Al) was able to accomplish regeneration at the end of day 14 (Fig. 3B). Moreover, 100% survival of the planarians was maintained in the control group for 14 days. Only 40% survival was observed in the group exposed to 400 mg/L Al at the end of day 14, whereas planarian exposed to 800 mg/L survived for only 4 days (Fig. 3C). Compared to the 400 mg/L exposed group, stereo-microscopic observations for the eye regeneration of the control group (water adjusted to pH 7.5 and 4.5; Fig. 4A, B) showed clear eye spot from days 5–14 (Fig. 4C). In the whole-mount immunohistochemistry observations, all three groups showed initiation of regeneration (Fig. 5A, D, G) and eye spots on day 5 (Fig. 5B, E, H). However, formation of the optic nerve was clearly seen only in the controls (Fig. 5C, F), as compared to planarian incubated with 400 mg/mL Al (Fig. 5I).
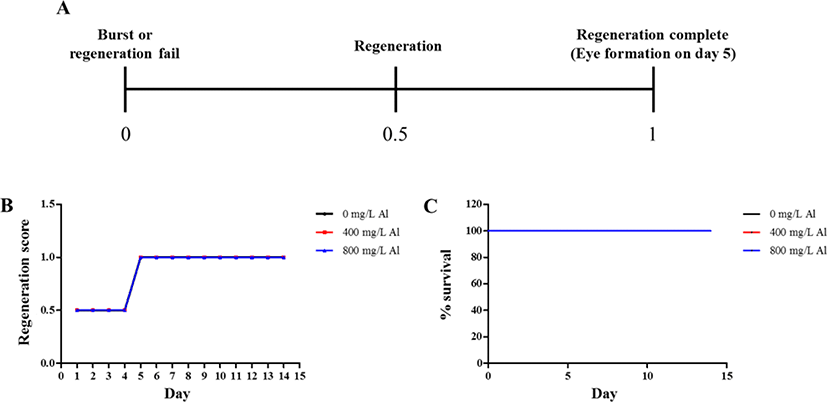
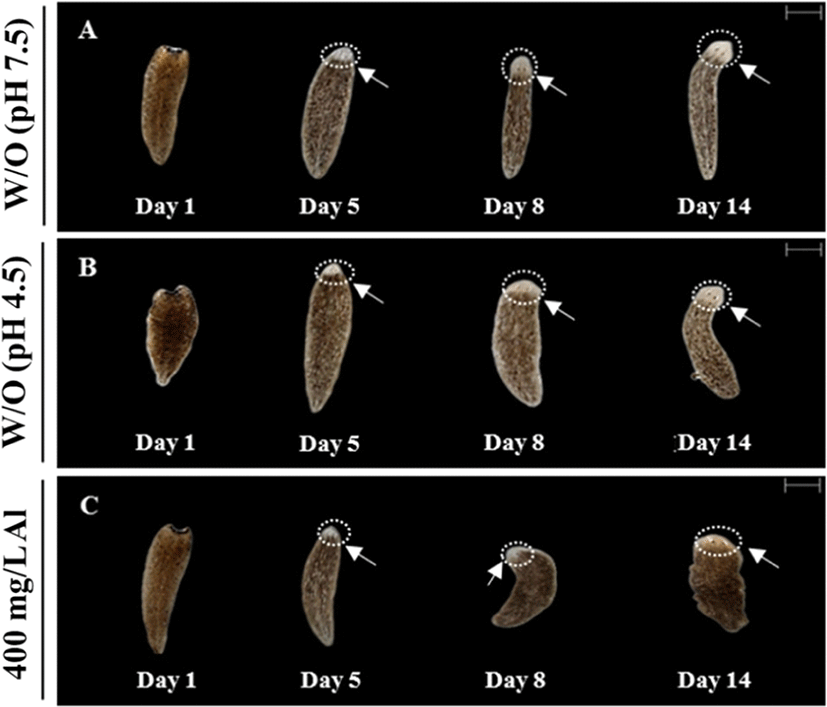
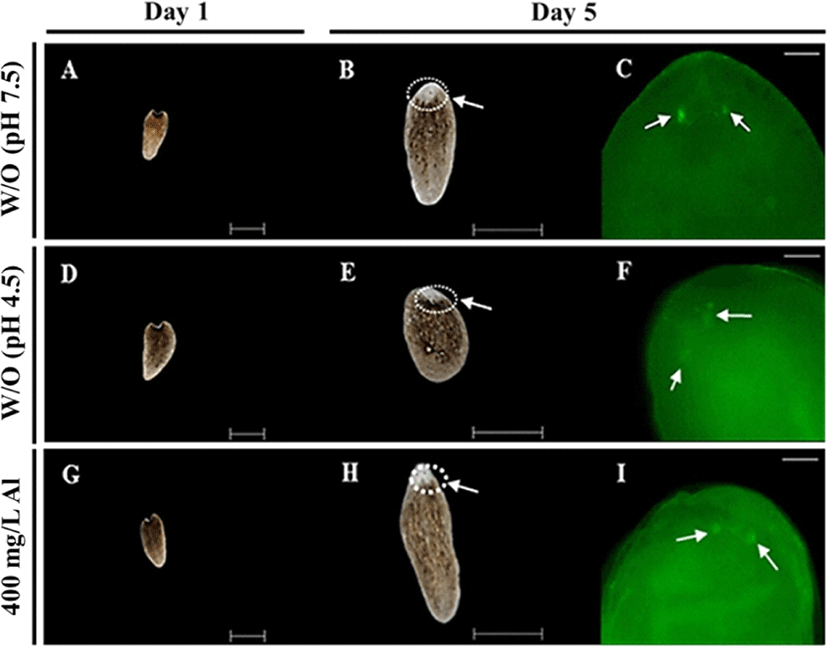
Examining the organelle analysis revealed that planarian cells treated with freshwater displayed regular mitochondrial structures (Fig. 6A), but the Al-exposed animals displayed irregular mitochondrial structures (Fig. 6A’). Emergence spaces between the membranes of nuclei were wider in the Al-treated group than in the control group. Compared to the control group, more empty spaces were observed within the membranes in the Al-treated group (Fig. 6B, B’). As presented in Fig. 6C and C’, an irregular endoplasmic reticulum (ER) structure was observed in the Al-treated group, as compared to the control groups. Although sections differed morphologically depending on their location, cilia of the Al-exposed planarians displayed more empty spaces than the fresh water groups (Fig. 6D, D’ and E, E’). Moreover, shrunken basal bodies (Fig. 6E, E’) and irregular golgi apparatus with bigger cisternae gaps (Fig. 6F, F’) were observed in the Al-treated group, as compared to the controls. The above obtained results indicate that exposure to Al causes morphological changes in organelles within the nerve system of planarians.
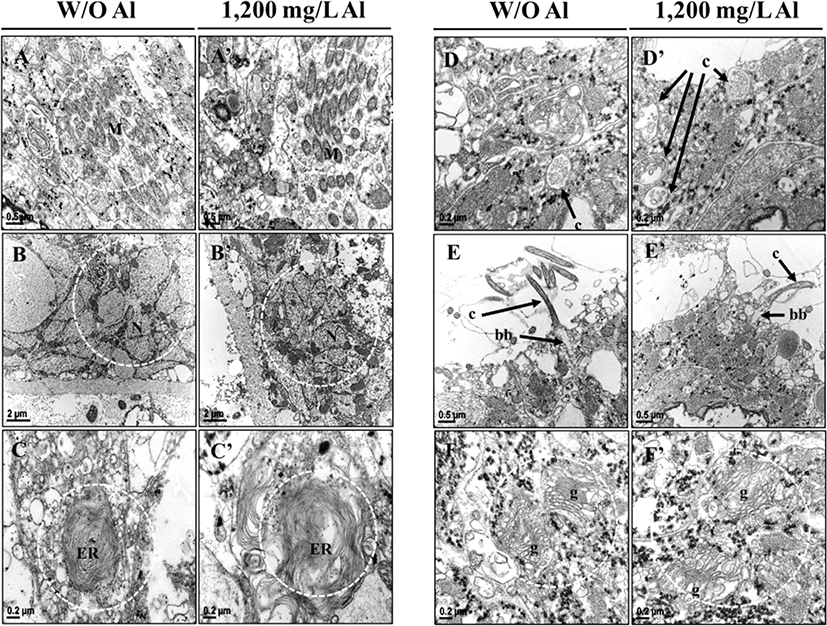
Discussion
Heavy metal toxicity is one of the emerging problems in the modern world, and studies regarding heavy metal toxicity have steadily received attention since 1946 [17]. Rapid industrialization leads to an increment in metal concentrations and pollution in the environment [2]. Heavy metals cannot be biodegraded, and are therefore accumulated in aqueous and terrestrial ecosystems [18]. These heavy metals are subsequently ingested through food, drinking water and air [19]. Accumulation of heavy metals induces toxicity which threatens all living organisms. The toxic effects of heavy metals have widely been published in previous experimental studies [20–22], with Al being reported as one of the common neurotoxicants [23]. According to Woodburn et al. [24], accumulation of Al causes inflammation in the hepatopancreas of fresh-water organisms; another study on aquatic invertebrates reported, lower survival rates with the increasing Al concentration [25]. Comparably, exposure to Al reportedly caused metabolic depression and oxidative damages in fresh-water invertebrates [26]. Accumulation of the Al induces the imbalance between reactive oxygen species production and antioxidant capacity in the cells. Those species are short lived but highly reactive and they will damage DNA as well as the macromolecules which are cytosolic and membrane-bounded. Moreover, Al has the potential ability to catalyze the formation of iron based oxidant events. Thereby, it will cause oxidative stress and apoptotic cell death or tissue damage [27]. Kapu and Schaeffer [28] stated that metal toxicity is related to concentration and exposure time. Similarly in the present study, normal behavior of the planarians was altered when they were exposed to different concentrations of Al for different incubation periods, whereas motility was decreased and seizure-like behaviors were increased in a dose-dependent manner. Moreover, decreased survival rates were observed in planarians exposed to the treatment solutions. Previous studies on planarians reported that heavy metals inhibit the regeneration abilities [29], and time taken to regenerate the eyespot and auricles was significantly longer in the treatment-exposed group than the groups exposed to water [30]. Likewise, delayed eye regeneration from tail fragments was observed in the Al-exposed planarians in the current study.
Most laboratory experiments were conducted using vertebrate animals [31–33], but issues related to high cost, rules and regulations is making this more difficult. Hence, there arises the necessity to discover an alternative animal model to replace vertebrate laboratory animals. The Dugesia japonica is a flatworm capable of regenerating body fragments after amputation, and possesses bilateral symmetry of the nervous system [34, 35]. The planarian nervous system is reminiscent of the vertebrate brain due to synaptic organization and morphological features. Besides, several common neurotransmitters have been identified in both planarians and humans [7]. The planarian brain is therefore suggested to being similar to the vertebrate brain evolution. Grebe and Schaeffer [36] used planarians in neurobehavioral toxicity screening assays and several other studies, and have reported the application of planarians to investigate locomotor activity and behavioral responses for drug effects on the nervous system [37–39]. In the current study, planarians exhibited sensitivity towards the varying concentrations of Al, and we were able to conduct regeneration observations within a short time period (14 days). The results of this study suggest that eye and nerve regeneration is related to heavy metal concentration and mitotic activity of neoblasts. Throughout the experiment, daily management practices and cost management were comparatively easier and more convenient with planarians. The above mentioned unique characteristics and preceding literature indicates the suitability of planarians as a potential alternative animal model for laboratory studies, especially those engaging in toxicity studies.