Introduction
Radioisotope ADME (RI-ADME) studies are performed to evaluate the in vivo absorption, distribution, metabolism, and excretion of radiolabelled compounds in the body [1]. Since numerous novel therapeutic antibodies have appeared in new drug development pipelines, antibodies labelled with positron-emitting radioisotopes or gamma ray-emitting radioisotopes can be used for acquiring quantitative images for the in vivo behaviour of drugs through molecular imaging. These antibodies are also widely applied in pharmacokinetic (PK) studies to quantify the distribution of drugs in target and normal organs [2–4].
Molecular imaging methods such as radiotracer-based single-photon emission computed tomography/computed tomography (SPECT/CT) can visualize the in vivo behaviour of radiolabelled compounds in a non-invasive manner. Particularly, in preclinical studies, imaging studies in live animals have many advantages. Real-time dynamics of the biodistribution of radiolabelled compounds can be obtained in the same animal and the number of animals used in the study can be minimized. Thus, these tools are essential for ADME evaluation of new drug candidates [2, 5, 6]. Radioisotopes used to radiolabel antibodies are typically composed of radiometals and radiohalogens. Metallic radionuclides such as 90Y, 111In, 177Lu and 99mTc can be used to label antibodies via coordination bonds with functional group of proteins or chelators [7, 8–10].
However, most monoclonal antibodies do not contain metal coordination sites, which reduces the stability of the antibody following metal radionuclide labelling. To overcome this obstacle, bifunctional chelators are attached to metal radionuclides. As metal radionuclides have varying coordination chemistries and properties for each radioisotope, a bifunctional chelator suitable for each isotope must be applied. Additionally, the coordination chemistry of metal radionuclides can affect the stability and geometry of radiometal chelators [8, 9, 11, 12].
Radiohalogens such as radioiodine, can directly label the tyrosine or histidine residues of monoclonal antibodies without chelator conjugation via stable covalent bonds. In addition, the steric and electronic properties of radiohalogens are expected to cause minimal modifications to proteins while showing very high specific activity [10, 11, 13, 14].
Radiohalogens are most often, particularly radioiodines, used to label antibodies since (1) the stable binding forms of radioiodine cause minimal changes and increase stability of the protein backbone, (2) the labelling methods are simple, (3) their chemistry is well characterized, (4) they allow for high radiochemical purity and specific activity, and (5) demonstrate a relatively long half-life. Hence, radioiodine labelling of biomolecules has been widely applied to studies, including those for screening new drug candidates in radio-immuno assays and preclinical ADME studies [7, 10, 13, 15–17].
The most commonly used radioiodines are 123I, 124I, 125I, and 131I [7]. Since 125I emits the lowest gamma ray energy (27–32 keV) and has the longest half-life (t1/2 = 59.4 days) among commonly used radioiodines, it is expected to cause minimal radiation damage to labelled antibodies. 125I is also the first radioisotope to be considered for preclinical biodistribution studies of radiolabelled antibodies due to its high specific activity, relatively low cost, well-characterized iodination chemistry, and long half-life [2, 14, 18]. Particularly, 125I is used for antibody labelling to reveal metabolic pathways in sacrificed animals by gamma-counter and is mainly used for PK, SPECT imaging, and in vivo studies [7, 19, 20]. Therefore, we selected 125I to evaluate its in vivo behavior and to perform imaging studies for up to 3 weeks in this study.
Trastuzumab (herceptin; Genentech, South San Francisco, CA, USA) is the first HER2-targeting recombinant humanized monoclonal antibody approved by the Food and Drug Administration for treating patients with HER2-overexpressing metastatic breast cancer. It significantly increases the survival rate of patients and has been used to treat early stage and metastatic breast cancers [21–23]. However, many patients show intrinsic resistance to trastuzumab. Additionally, patients showing clinical effects early in treatment have experienced disease progression within one year of treatment because of the development of resistance to long-term treatment [24–26].
Recently, many targeted therapy studies have been conducted to treat cancer more effectively and safely. In this type of therapy, specific targeted molecules selectively act only on cancer cells to block their rapid division [27]. An antibody-drug-conjugate (ADC) is a structure that combines a monoclonal antibody with cytotoxic payload using a linker and has shown excellent therapeutic effects in clinical trials [28–30].
Currently, tubulin inhibitors (MMAE, MMAF, DM1, DM4) are widely used as ADCs with cytotoxic payload delivery to tumor tissues. Particularly, monomethyl auristatin (MMAE, MMAF) is the most commonly used and is applied in nearly 50% of clinical studies. Monomethyl auristatin F (MMAF) is used as cytotoxic payload in nine ADCs, including ADCs targeting the HER2 antigen, and is actively being evaluated in clinical studies [31–33]. MMAF is a very stable molecule that is not degraded in the plasma, human liver lysosomes, and by proteases such as cathepsin B [34] and shows excellent efficacy and high stability in ADCs [35]. The test substance LCB14-0110 used in this study is an ADC with indications for HER2-positive breast cancer. LCB14-0110 is comprised of MMAF conjugated to trastuzumab.
Two animal species were used for RI-ADME studies. Xenograft model mice without immune cells into which patient-derived tumors or cell lines are transferred are commonly used in preclinical studies to examine the anticancer effects of drugs [36, 37]. The common marmoset monkey (Callithrix jacchus) is a small non-human primate (NHP) that is more similar to humans than to rodents in its anatomical structure, genetic and physiological functions, and metabolism. Compared to larger NHPs, it has a large body surface area, which requires a rapid metabolic rate and small amount of test substance, thereby saving both time and money. Thus, marmosets are being increasingly used in preclinical studies [38–40]. In fact, marmoset monkeys have been evaluated in PK (ADME) studies, in toxicity evaluations of new drug candidates, cognitive studies, and pharmacodynamics analyses (neuroscience, immunology, infectious disease) [40, 41].
In this study, after labelling LCB14-0110 with 125I to minimize antibody structural alterations, gamma-counter and SPECT/CT imaging were performed to evaluate the human breast cancer tumor targeting and biodistribution of 125I-LCB14-0110 in HER2-positive breast cancer cell-bearing mice. In addition, we evaluated the biodistribution and accumulation of 125I-LCB14-0110 in normal organs in healthy marmoset monkeys.
Materials and Methods
LCB14-0110 was used as a trastuzumab-MMAF and provided by LegoChem Biosciences (Daejeon, Korea). As reagents for radioisotope labelling, chloramine-T and sodium metabisulfite were obtained from Sigma Aldrich (St. Louis, MO, USA). [125I]NaI was purchased from Saehan Industry (Seoul, Korea). High-purity tertiary distilled water (deionized water, 18.2 MΩ, Millipore, Billerica, MA, USA) was used. A plate for radio-thin-layer chromatography (TLC; Silica gel 60 F254-25 aluminum sheets 20 × 20 cm) was purchased from Merck (Darmstadt, Germany).
For the radiolabelling, modified Ma’s method was used [42]. Briefly, LCB14-0110 was directly labelled with radioiodine by electrophilic aromatic substitution of tyrosine residues without chelator conjugation. Chloraimine-T (10 μL, 30 μg in phosphate-buffered saline; PBS) and 1 mCi of [125I]NaI were added to 1 mL of LCB14-0110 (0.15 mg/mL). The reaction was performed at room temperature for 90 s. Next, 10 μL of sodium metabisulfite solution (90 μg in PBS) was added to quench the radioiodine reaction. The radiolabelled drug was purified using a PD-10 column. The developing solvent was acetone. The radiochemical purity was determined by radio-TLC analysis (AR-2000, BIOSCAN, Poway, CA, USA).
The human breast cancer cell line JIMT-1 (HER2-positive) was purchased from Addexbio (San Diego, CA, USA). JIMT-1 cells were incubated in Dulbecco’s Modified Eagle Medium containing 1% antibiotics + pyruvate (Gibco, Grand Island, NY, USA) and 10% fetal bovine serum. Subcultures were performed every 3–4 days at a 1:5 ratios. The cells were incubated in a humidified atmosphere containing 37°C and 5% CO2.
To evaluate the in vitro stability of 125I-LCB14-0110, experiments were performed in PBS, mouse serum, and human serum. First, 100 μL of 125I-LCB14-0110 (30 μCi) was added to 400 μL of PBS, mouse serum, and human serum and then reacted at room temperature for 0, 1, 3, 7, 14, and 21 days. Stability analysis was performed over time by radio-TLC.
All animal studies were approved by the Osong Medical Innovation Foundation Animal Care and Use Committee (KBIO-IACUC-2019-047 & 048) and were conducted in accordance with the guidelines of the committee.
Five-week-old female BALB/c-nude mice were purchased from Orient Bio (Gyeonggi, Korea). The mice were inoculated subcutaneously into the right flank with 0.2 mL solution containing 2 × 106 JIMT-1 cells in PBS (100 μL) with 100 μL of Matrigel (Corning, Corning, NY, USA). When the mean tumor volume reached approximately 200 mm3, the animals were used for in vivo studies.
The blood PK of 125I-LCB14-0110 was evaluated in JIMT-1 tumor-bearing mice (n = 3/time point). Blood was collected at each time point (1 and 6 h, 1, 3, 7, 14, and 21 days) after intravenous administration 2 μCi (154 ng/mice) of 125I-LCB14-0110 to xenograft mice.
Phoenix® WinNonlin® 8.0 version, Certara, St. Louis, MO, USA) was used for noncompartmental PK analysis. The half-life (t1/2), clearance (CL), volume of distribution (Vd), area under the blood concentration-time curve from time zero to the last measurable concentration (AUClast), and area under the blood concentration-time curve from time zero to infinity (AUCinf) were calculated. PK parameters values are expressed as the mean ± S.D.
To assess the biodistribution of 125I-LCB14-0110 in JIMT-1 tumor-bearing mice, samples of major organs (heart, spleen, liver, stomach, kidney, intestine, lung, thyroid) and tumors were harvested following sacrifice under inhalation anesthesia (1%–2% of isoflurane in 1 L/min of oxygen) at each time point (1 and 6 h, 1, 3, 7, 14, and 21 days).
Radioactivity in each organ was measured with a gamma-counter (2480 WIZARD2, PerkinElmer, Waltham, MA, USA). Biodistribution results were expressed by calculating the percentage of the injected dose per gram of tissue (%ID/g, mean ± S.D.).
After intravenous administration of 400 μCi 125I-LCB14-0110 (30 μg/head) in JIMT-1 tumor-bearing mice (n = 2), SPECT/CT images were obtained using small animal SPECT/CT (Nano PET/CT, Mediso, Budapest, Hungary). Under inhalation anesthesia (1%–2% of isoflurane in 1 L/min of oxygen), CT images were acquired to obtain anatomical information. SPECT images were acquired at different time points (1 h, 1, 3, 7, 14, and 21 days), and whole-body images were obtained with 20% energy windows using a multi pinhole collimator.
The biodistribution of 125I-LCB14-0110 was evaluated in female healthy common marmoset (n = 2, body weight = n1: 304, n2: 298 g). Blood was collected from the femoral vein at one day after intravenous administration of 10 μCi 125I-LCB14-0110 (0.77 μg/head). Subsequently, the marmosets were sacrificed under anesthesia, and major organs (heart, spleen, liver, stomach, kidney, intestine, lung) were collected. Biodistribution study was carried out as described above for tumor-bearing mice.
After intravenous administration of 700 μCi 125I-LCB14-0110 (54 μg/head) to healthy common marmoset female monkey (n = 2, body weight = 369(n1), 327(n2) g), SPECT/CT images were obtained by small animal SPECT/CT (Nano PET/CT, Mediso). Cardiac anesthesia was induced by intravenous administration of 30 mg/kg zoletil and 10 mg/kg rompun before SPECT/CT imaging. Under inhalation anesthesia (2%–2.5% of isoflurane in 1 L/min of oxygen) CT images were acquired for anatomical analysis. SPECT images were taken at different time points (1 h, 1, 3, 7, 14, and 21 days), and whole-body images were obtained with 20% energy windows using a multi pinhole collimator.
Results
Radio-TLC was performed to confirm labelling of LCB14-0110, with acetone used as the developing solvent. Free 125I showed a retention factor (Rf) of 0.99 and radiolabelled 125I-LCB14-0110 had an Rf value of 0.01. The radiochemical purity was 100% (Fig. 1).
To evaluate the stability of 125I-LCB14-0110, 100 μL of 125I-LCB14-0110 (30 μCi) was added to 400 μL of PBS, mouse serum, and human serum and allowed to react at room temperature for 3 weeks. The reactions were analysed over time by radio-TLC.
125I-LCB14-0110 showed high stability of approximately 100% in PBS, mouse serum, and human serum up to day 3. On day 7, stability in PBS was 98.71%, in mouse serum was 99.08%, and in human serum was 100%. On day 14, stability values were 97.24%, 98.11%, and 98.65%. on day 21, these values were 94.86%, 98.7%, and 98.03%, respectively.
Free 125I accounted for less than 5% of the total 125I-LCB14-0110 over three weeks (Fig. 2), and no significant difference over time was observed. 125I-LCB14-0110 showed high stability in vitro.
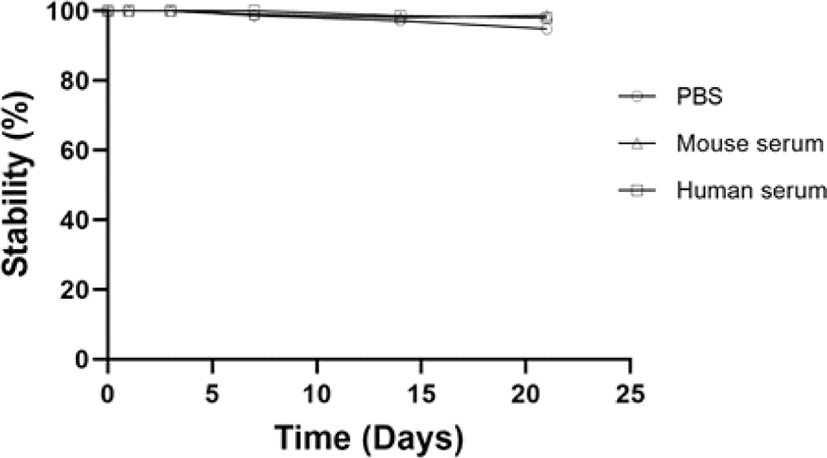
The blood PK parameters of 125I-LCB14-0110 were calculated by collecting blood at different time points (1 and 6 h, 1, 3, 7, 14, and 21 days) after intravenous administration of 125I-LCB14-0110 in JIMT-1 human breast cancer xenograft mice (Fig. 3). The blood PK parameter was calculated from the average value of the sampling time point using three animals at each time point. The results showed the highest radioactivity at 1 h after administration (39.06 ± 8.08% ID/g). After this time, the radioactivity was distributed to organs over time, showing a 99% reduction at 0.46 ± 0.19% ID/g on day 21.
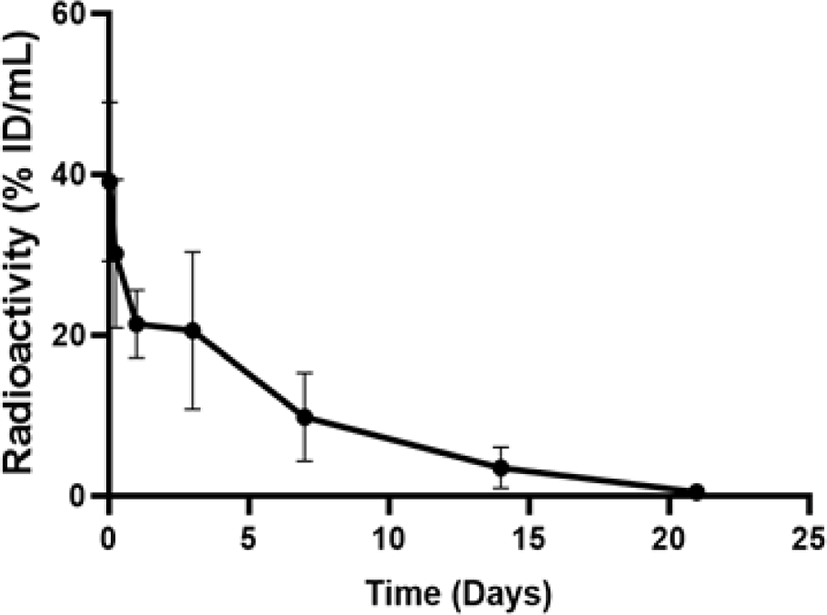
Blood PK parameters are shown as the half-life (t1/2): 82.71 h, area under the concentration-time curve from time zero to the last measurable concentration (AUClast): 4,331.65 (% ID × h/mL), area under the concentration-time curve from time zero to infinity (AUCinf): 4,374.46 (% ID × h/mL), volume of distribution: 4.20 (mL/kg), and CL: 0.035 (mL/h/kg).
The biodistribution was evaluated for 21 days after intravenous administration of 2 μCi 125I-LCB14-0110 (154 ng/mouse) in HER2-positive JIMT-1 cell-bearing mice with a gamma-counter. As a result, the tumor showed the highest uptake at day 3 which increased by approximately 300% compared to at 1 h (1 h: 3.23 ± 1.41% ID/g, day 3: 12.96 ± 5.36% ID/g). We observed increased uptake into the tumor for up to 3 days, followed by a decrease from 7th day (Fig. 4). The kidneys and lungs in normal organs showed relatively high radioactivity at 1 h (9.37 ± 3.43% ID/g, 14.25 ± 3.55% ID/g, respectively), but showed lower radioactivity than the tumors at day 1 (kidney: 4.61 ± 0.88 %ID/g, lung: 8.09 ± 0.74 %ID/g, tumor: 9.22 ± 0.75% ID/g) and so on. At 6 h, radioactivity was increased in the spleen, stomach, and intestine compared to at 1 h, but thereafter showed a sharp decrease over time.
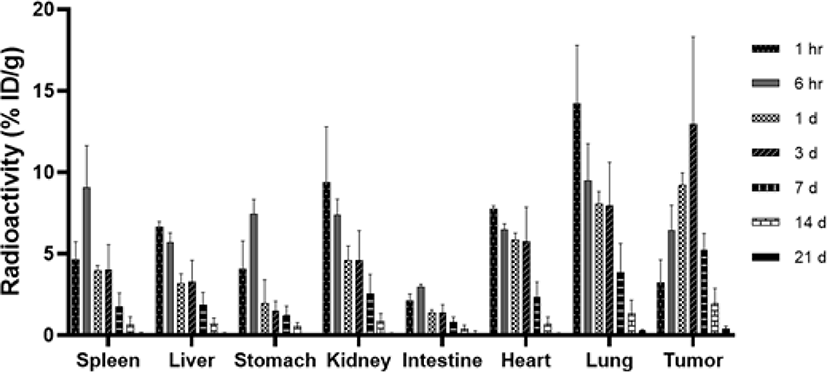
Comparing the radioactivity of tumor and blood (Fig. 5), LCB14-0110 was targeting to the tumor and the tumor to blood ratio (T/B ratio) was gradually increased over time.
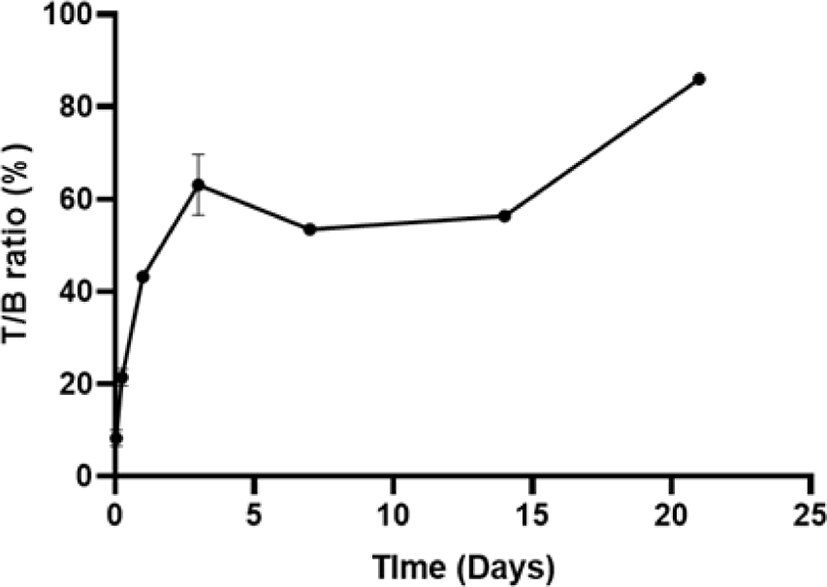
Small animal SPECT/CT images were acquired at 1 h, 1, 3, 7, 14, and 21 days after intravenous administration of 400 μCi 125I-LCB14-0110 (30 μg/mouse) in HER2-positive JIMT-1 cell-bearing mice (Fig. 6).
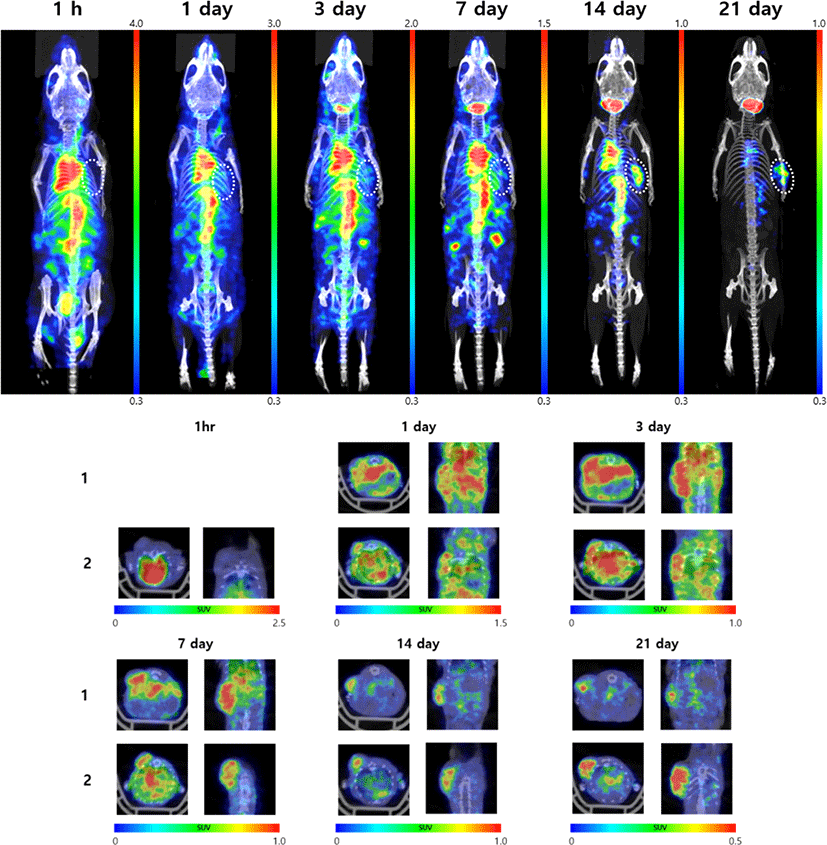
Uptake was high in the heart, liver, lung, and kidney at 1 h, and then decreased over time. On 14th day, 125I-LCB14-0110 had lost much radioactivity in the whole body. On 21th day, nearly no radioactivity remained in the body except for in the thyroid and tumor. The tumor showed increased uptake over time starting on day 1, with the highest uptake and accumulation found on day 3.
To evaluate the biodistribution of 125I-LCB14-0110 in normal animals, 10 μCi (0.77 μg/head) of 125I-LCB14-0110 was intravenously administered to healthy marmosets. After blood was collected on day 1, sacrificed marmosets were assessed for biodistribution study using a gamma-counter (Fig. 7). As a result, the highest uptake was found in the spleen (n1: 2.31, n2: 4.22% ID/g), and other organs showed low radioactivity of less than approximately 1% ID/g.
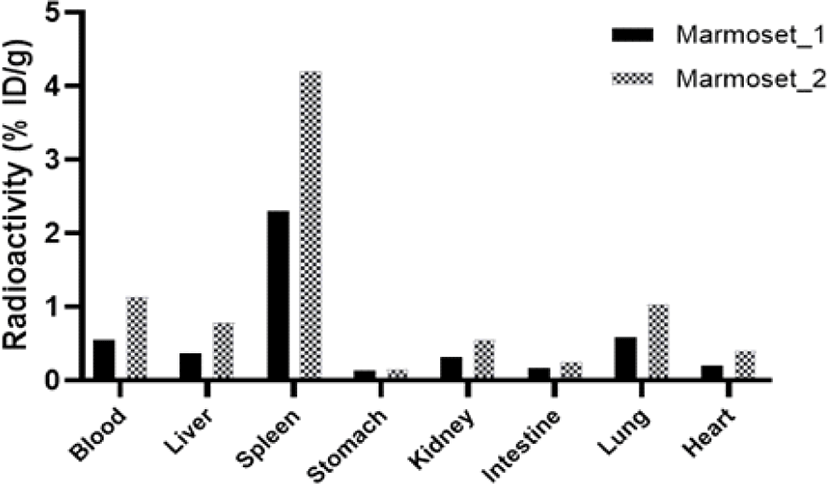
Whole-body small animal SPECT/CT images were acquired at 1 h, 1, 3, 7, 14, and 21 days after intravenous administration of 700 μCi 125I-LCB14-0110 (54 μg/head) in healthy marmosets. Relatively high uptake was observed in the heart, liver, stomach, kidney, and spleen at 1 h (Fig. 8). Except for the thyroid gland, whole-body radioactivity rapidly decreased starting at 3 days, and most radioactivity administered was excreted within 1 week. The results confirmed no radioactivity accumulation in the normal organs.
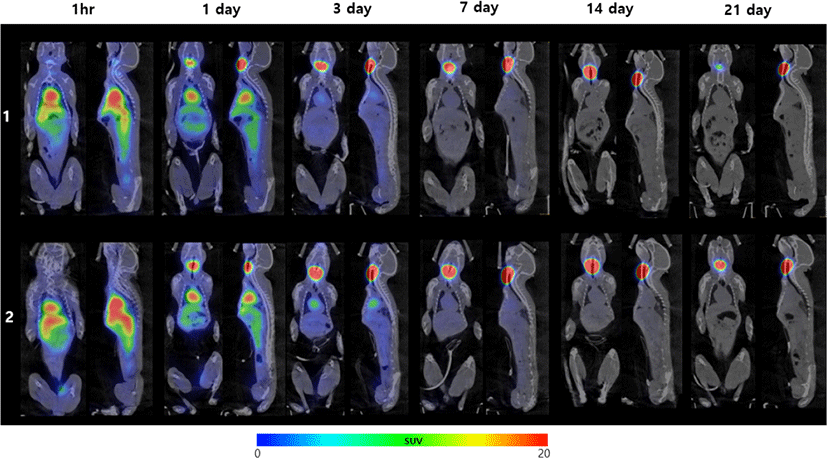
Based on the biodistribution results described in section 3.3.1.2 tumor-bearing mice and section 3.3.2.1 in healthy marmoset, the distribution of radioactivity in the normal organs in the two animal species at day 1 after 125I-LCB14-0110 administration was compared. We calculated the % ID/tissue considering the difference in organ weight of the mice and marmoset (Table 1). The xenograft mice showed higher distribution in the blood and intestine than marmosets. Whereas, the radioactivity of spleen and liver of marmoset was higher than those of tumor-bearing nude mice. Other organs showed similar distributions in the two animal species.
Discussion
After labelling radioiodine with antibodies to minimize modification of the antibody structure in LCB14-0110, we evaluated the tumor targets and in vivo behavior by gamma-counter and SPECT/CT imaging. In this study, 125I-LCB14-0110 showed a high radiochemical purity and high stability for 3 weeks in both mouse and human serum. Based on these results, radioiodine labelling of the ADC in this study was useful for in vivo imaging and long-term PK studies. Therefore, in vivo studies were conducted. To evaluate the tumor targeting ability and in vivo behavior of 125I-LCB14-0110 (HER2 Targeting anticancer agent), PK analysis and biodistribution studies in tumor-bearing mice and healthy marmoset monkeys were performed by gamma-counter, with in vivo behavior images obtained by using SPECT/CT. Xenograft mice and marmosets were administered intravenously with specific activity (13 μCi/1 μg) for using optimized dose in biodistribution study and SPECT/CT imaging. Stollman et al. reported that addition of unlabelled antibodies to labelled antibodies significantly reduced the amount of labelled antibody targeted to tumors when adjusted to the clinical dose [43]. Accordingly, we used the optimal radioactivity dose rather than the clinical dose of 125I-LCB14-0110 to confirm tumor targeting.
Biodistribution evaluation using a gamma-counter in tumor bearing mice showed the highest uptake and accumulation at day 3, with radioactivity increasing over time in the tumor. Additionally, increasing the T/B ratio may have greatly affected the tumor-targeting ability.
In normal organs of tumor-bearing mice, uptake by the lung and kidney was relatively high at 1 h. At 6 h, increased radioactivity was observed in the spleen, stomach, and intestine compared to at 1 h. Healthy marmosets showed the highest uptake in the spleen (n1: 2.31, n2: 4.22% ID/g) at day 1. Increasing radioactivity in the stomach and intestine of tumor bearing mice has previously been reported for gastric radioiodine accumulation which occurs because of the in vivo catabolism and deiodination of labelled antibodies when using iodine direct labelling [7, 44]. Accordingly, we determined that transiently high uptake in the stomach and intestines occurred because of radiolabelling.
In addition, the lung, spleen, and kidney of tumor-bearing mice showed high radioactivity. This results were similar to those of other studies in which trastuzumab was administered to HER2-positive tumor-bearing mice [45–47]. According to Yang et al. [46], trastuzumab showed high uptake in the spleen and kidneys, which are organs of the reticuloendothelial system (RES) and detects external substances based on sufficient blood flow and metabolism. Additionally, the lungs are generally known to exhibit acute accumulation after trastuzumab administration and showed temporary high uptake of radioactivity in this study [48]. Other normal organs and blood showed continuous decreases in radioactivity over time.
Small animal SPECT/CT imaging revealed high uptake in the heart, liver, lung, and kidney at 1 h post-administration of 125I-LCB14-0110 in tumor-bearing mice. These results are consistent with the high uptake by normal organs at the same time point in the biodistribution study. SPECT/CT imaging showed a rapid decrease in radioactivity at day 14, with very low radioactivity in the whole body except for in the tumor and thyroid areas on day 21. Whereas radioactivity in the whole body and major organs decreased over time, in tumors, radioactivity increased gradually until day 3, showing the highest uptake at this time point. Intratumoral radioactivity was observed until 21 days, confirming tumor targeting and accumulation. The biodistribution results were similar to the tumor SPECT/CT imaging results and to those of another study of the correlation between SPECT/CT imaging and biodistribution [49], confirming the relation between the biodistribution and SPECT/CT imaging of 125I-LCB14-0110 in tumor-bearing mice. Our results confirmed the tumor targeting profile and distribution profile in normal organs.
Marmosets showed higher uptake of radioactivity in the spleen than in the other normal organs. Similar results were obtained from other studies comparing the biodistribution of 64Cu-labelled trastuzumab in mice with HER2-positive breast tumors and normal mice. The results showed that in normal mice, the spleen had the highest uptake of radioactivity among normal organs [50]. Clinical studies showed similar results. Whole body PET imaging results at 1, 24, and 48 h after injection 64Cu-1,4,7,10-tetraazacyclododecane-1,4,7,10-tetraacetic acid-trastuzumab in HER2-positive breast cancer patients revealed significantly higher accumulation in the spleen at all-time points. These results indicate that monoclonal antibodies interact with specific antigens or are specifically captured by splenic macrophages, causing accumulation in the spleen [51, 52].
SPECT/CT imaging results in marmosets showed relatively high radioactivity in the heart, spleen, liver, stomach, and kidney on day 1. The difference from the biodistribution results was less than 3% ID/g. The similarity of uptake in normal organs was confirmed in the biodistribution study and SPECT/CT imaging results. Particularly, SPECT/CT imaging showed high uptake in the heart at 1 h. Radioactivity in the heart, where large blood vessels are located, was higher than that observed by gamma counting, which revealed radioactivity after tissue extraction and perfusion [53]. After 3 days of administration, whole-body radioactivity except for in the thyroid was rapidly reduced, with no accumulation in normal organs.
Comparison of the distribution of radioactivity in normal organs between two animal species at the same time point (1 day post-administration) showed that LCB14-0110 had a far lower spleen uptake (more than 13 times lower considering organ weights) and relatively higher (1.8 fold) blood radioactivity in nude mice than healthy marmosets. These differences may be caused by the role of filtering the antigen in the spleen was reduced due to lack of immunity. Accordingly, the overall loss of radioactivity is also observed more rapidly in the marmoset.
In addition, T-DM1 was found to be toxic in the spleen of Sprague-Dawley rats and cynomolgus monkeys and was accompanied by RES hypertrophy as well as lymphocyte necrosis and degeneration [54], with similar results found in clinical studies. Magnetic resonance imaging of patients with metastatic breast cancer treated with T-DM1 indicated an increase in the average spleen size from 144 cm3 (95% CI 110–177 cm3) to 209 cm3 (95% CI 161–257 cm3) in 92% of patients [55]. This indicates that the conjugated drug of ADC is degraded in the antibody and then released and accumulates in the spleen [56]. The high radioactivity in the spleen of marmosets in this study was thought to occur for this reason. Whereas, relatively low distributions were observed in other normal organs of marmosets.
Comparison of the results of clinical studies of trastuzumab and biodistribution of LCB14-0110 marmosets revealed similarities, with higher uptake in the spleen. As described by Smith et al and Hart et al. [39, 57], marmosets have similar metabolism and immune systems as humans, and thus show similar biodistributions of drugs. Therefore, marmosets are useful for predicting the human biodistribution of test substances in ADME research in the new drug development process.
We confirmed the effective tumor targeting and accumulation of 125I-LCB14-0110 in HER2-positive tumor-bearing mice and verified continued reduction of radioactivity in the blood and normal organs over time. Radioactivity rapidly decreased in healthy marmosets without targeting normal organs. Additionally, the distribution of each organ of 125I-LCB14-0110 in mice and marmoset was similar except spleen. In conclusion, 125I used is expected to be a useful tool in the study of RI-ADME in biopharmaceuticals through minimal antibody modification.