Introduction
A challenging aspect of pharmacological and/or toxicological research has been the identification of cellular processes in live animals. Among these processes, cell death could serve as an indicator for chemical-induced change(s) [1]. Practically, cell death cannot be observed by the naked eye in live animals, nor by stereomicroscope during necropsy. As estimating cell death is a critical step in the evaluation of disease progression [2], many tools and techniques have been developed to study it [3–8]. Among them, histopathological techniques, such as general staining or specific immunohistochemical staining, have a strong advantage for the detection of cell death that occurs in a cell specific manner [9]. However, these conventional techniques take more time to identify cellular damage than new approaches, such as bioimaging.
Developments in bioimaging technologies and techniques have led to their widespread use in toxicity studies of hazard chemicals and pharmaceuticals in development. Bioimaging is also increasingly used to visualize biological processes underlying disease activity [10]. For example, bioimaging has successfully monitored the progression and regression of pathological lesions [11]. Optical bioimaging using fluorescence probes has proved especially effective for visualizing biological processes [12].
Acetaminophen (APAP) is a common over-the-counter medication used for its analgesic and antipyretic properties [13]. APAP is mainly metabolized in the liver, and its use is often avoided in patients with chronic liver disease, owing to the risk of APAP-induced hepatotoxicity [14].
As bioimaging technologies have been used to provide quantitative data about pathological lesions [15], this study aimed to investigate whether fluorescence bioimaging could provide a readout of cell death in mice treated with APAP, and if so, to compare the results with those of conventional clinical and histopathological approaches.
Materials and Methods
Five-week-old, male ICR mice were obtained from Nara Biotech Co. Ltd. (Pyeongtaek, Korea) and acclimated for one week before the start of the study. The animals were placed in a temperature-controlled room (22 ± 3°C) with 55 ± 5% relative humidity and a 12-h light/dark cycle. They were fed with chlorophyll-containing ingredients, particularly, an alfalfa-free diet (Harlan Laboratories, Inc., Madison, WI) and filtered water. This study (NSU-15-6) was approved by the animal experiment committee of Namseoul University based on the Animal Protection Act.
At six weeks of age, eighteen mice were randomly divided into three groups. Group 1 (G1) and group 2 (G2) mice were treated with 0.9% saline in equal volumes at six weeks of age. Group 3 (G3) mice were orally treated with APAP (800 mg/kg b.w.) at equivalent times. Twenty two hours after APAP treatment, G2 and G3 mice were intravenously treated with the Annexin-Vivo 750 probe (PerkinElmer, Waltham, MA; 100 μL/mouse), according to the manufacturer’s instructions. Annexin-Vivo 750 is a targeted fluorescence imaging agent developed to detect molecular aspects of cell death [3]. At the same time, G1 mice were treated with equal volumes of 0.9% saline solution.
At twenty four hours after APAP treatment, the mice were anesthetized with 2.2.2-tribromoethanol (Sigma-Aldrich, St. Louis, MO). The mice were then placed horizontally in the imaging chamber of a fluorescence-labeled organism bioimaging instrument (FOBI) system (Neoscience, Suwon, Korea). Fluorescence bioimaging was conducted, and fluorescence position and intensity were recorded. The livers of the mice were excised, fluorescence intensity of the region of interest (ROI) was measured, and the images were analyzed. As fluorescence intensity decreases over time, measurement of signal intensity in the fluorescence probe control group was carried out within four hours after animal sacrifice.
After fluorescence bioimaging, blood was taken from the abdominal aortas of the mice, and serum levels of aspartate aminotransferase (AST), alanine aminotransferase (ALT), alkaline phosphatase (ALP) and lactate dehydrogenase (LDH) were analyzed.
After fixation in 10% neutral phosphate-buffered formalin, liver tissues were processed and embedded in paraffin, and 4 μm sections were stained with hematoxylin and eosin (H&E) for histopathological examination.
Tissue sections were dewaxed with xylene and hydrated. They were treated with proteinase K and 3% hydrogen peroxide, and with TdT enzyme and anti-digoxigenin peroxidase conjugate in In Situ Apoptosis Detection Kit (Chemicon International, Temecula, CA). Avidin-biotin complexes were visualized by DAB (Sigma).
Results
In vivo fluorescence intensity was detected in the livers of G2 and G3 mice, while those of G1 mice showed no signal, showing significant increase of fluorescence intensity of G2 and G3 mice compared to G1 mice (p<0.05 and p<0.01) (Fig. 1). Ex vivo fluorescence intensity of the livers from G2 and G3 mice was significantly increased compared with those of G1 mice (p<0.05 and p<0.01) (Fig. 2). However, in vivo and ex vivo data, there were no statistical difference of intensity between G2 and G3.
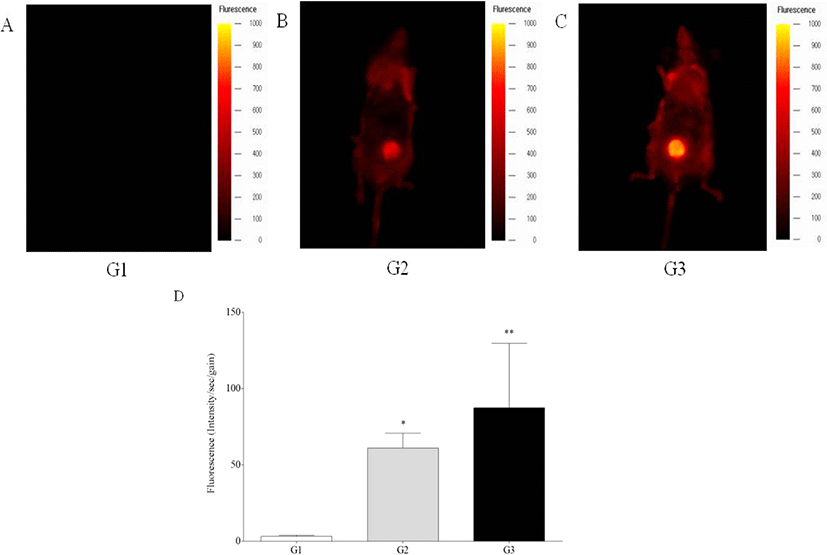
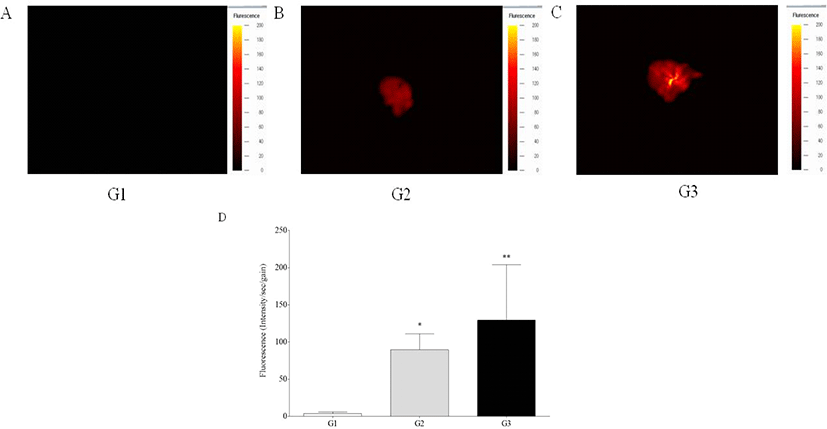
Serum levels of AST, ALT, ALP, and LDH were significantly increased in G3 compared to G1 and G2 (p<0.05 and p<0.01). However, there was no difference between G1 and G2 (Fig. 3).
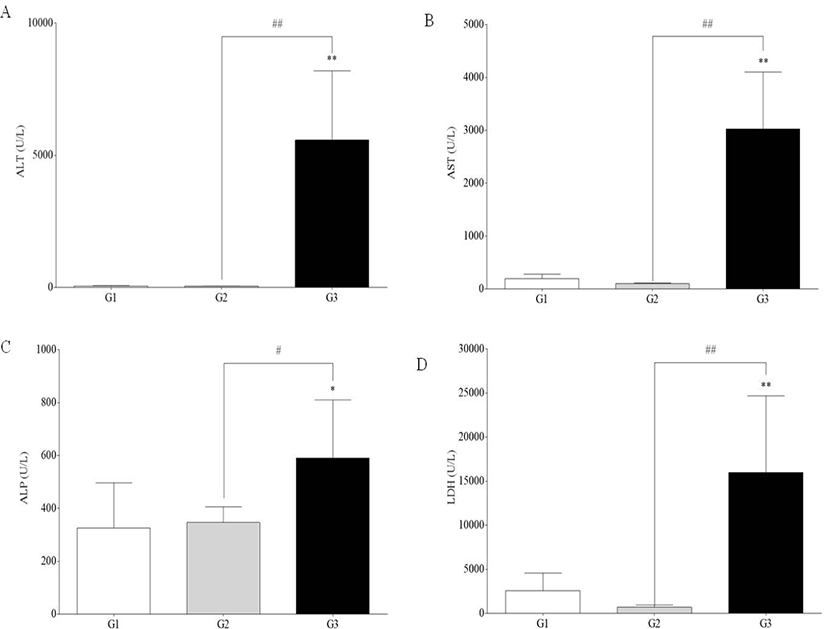
G1 and G2 mice showed no cell death in the liver. However, G3 mice showed acute hepatic cell necrosis, mainly located around the central veins of the livers (Fig. 4). Diffuse hemorrhage and infiltration of inflammatory cells and disruption of hepatic cell cords were also observed in the livers of G3 mice.
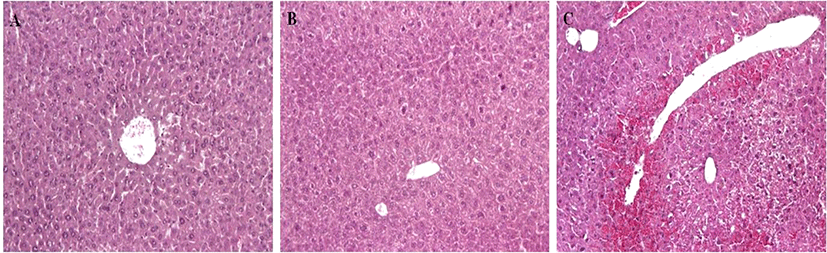
TUNEL staining showed many cell death features for G3, and none for G1 and G2 mice (Fig. 5). Numerous cell deaths were observed in the livers of G3 mice treated with APAP, mainly located around the central veins of the livers as shown in H&E.
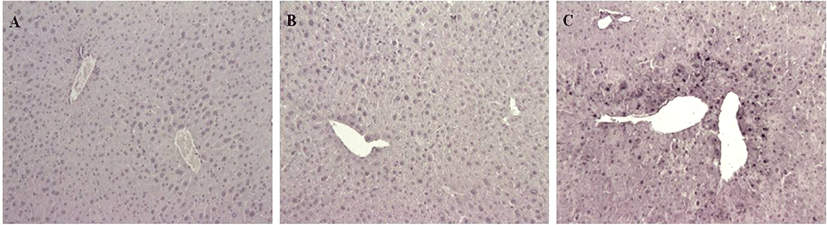
Discussion
Fluorescence bioimaging was used to visualize the target molecule for cell death. To investigate disease progression, it is important to detect and monitor pathological lesions in vivo. In this study, in vivo fluorescence bioimaging was used to visualize the target molecules for cell death, and the results generally agreed with clinical and pathological assessment. Specifically, in vivo fluorescence bioimaging showed that a significant increase in fluorescence intensity in G3 mice compared to G1 mice, representing effective quantification of cell death in the ROI. However, because of the depth limitation of fluorescence bioimaging [16], it was difficult to detect fluorescence signal in intact mice. Furthermore, fluorescence bioimaging did not provide clear discrimination of the liver from surrounding organs. Thus, for accurate quantification, it seems that a modality and/or software should be developed to anatomically distinguish the target organ(s) from surrounding organs.
This study’s in vivo imaging of cell death in mice using Annexin-Vivo 750 probe treatment confirms two of the advantages fluorescence bioimaging has over conventional histopathological examination: it allows both for in vivo tracing of altered biological processes, and for in vivo detection of specific molecule(s). However, the study also calls attention to some of in vivo bioimaging’s limitations. So, in this study, after removal of the skin and peritoneum of mice, in vivo bioimaging was obtained. With ex vivo imaging, it seems it is possible to detect the apoptotic area at a closer distance and lower background level. Ex vivo imaging is also limited, however, in that it does not provide sharp margins for damage cells.
Even though several near-infrared fluorescent imaging probes have been used for in vivo bioimaging [12, 17], this paper did not present probe control data, as it is difficult to differentiate probe-induced effect and from drug-induced effects and presents the limitations of fluorescence bioimaging, which will be very helpful for further investigations of bioimaging.
Serum chemistry data showed APAP induced cell damage in G3 mice and HE examination clearly showed G3 mice had severe hepatic damage. In particular, hepatic cells in centrilobular areas were more severely damaged than those in periportal areas in the livers of G3 mice. APAP is metabolized by sulfation and glucuronidation by CYP2E1, producing a reactive metabolite, N-acetyl-p-benzoquinone imine, which is detoxified by conjugation with glutathione [14]. APAP treatment in mice induces an acute phase of hepatitis with alteration of many genes, as shown in a previous report [18].
In this study, fluorescence bioimaging detected cell death in the livers of mice treated with APAP. This outcome was verified by serum chemistry and histopathological examination. However, in contrast to the HE and TUNEL applied in the study, fluorescence bioimaging was not able to clearly identify and differentiate specific pathological lesions in the liver. So, convention serum chemistry and histopathological tools showed cell damage clearly compared to fluorescence bioimaging in this study. The development of new techniques is necessary to over these limitations to current imaging methods [19–22]. Specifically, investigations into the detection of pathological lesions using bioimaging with high cellular resolution would be helpful.